The Search for the Missing Universe
Half of creation seems to be missing. We know it should exist, so where is it?
Anderson couldn’t explain what he was seeing. Most of the particles obeyed the laws of physics, flowing as predicted. But, scattered in the results, a handful moved in the wrong direction. Perhaps there was a mistake in his apparatus, or perhaps something strange was happening as they crashed through a sheet of lead placed to slow them down. But if not, if the results were correct, then Anderson might have found something unbelievable.
A few years earlier, an Austrian physicist, Victor Hess, had discovered fast-moving particles raining down on the Earth. These particles seemed to come from deep space, far beyond the realm of the Solar System. The discovery was fascinating, and drew the attention of the Nobel laureate Robert Millikan. For years he investigated the mysterious visitors, naming them cosmic rays, and entered into a fierce public debate about their origins.
As the leading scientist at Caltech, Millikan could draw upon large resources to support his research. He formed a team of researchers to assist him, and in 1930 he had hired Carl Anderson to build him a cloud chamber. This device, essential for tracking tiny high-speed particles, is a large box or sphere filled with air just on the edge of forming a cloud or mist. As a particle zips through the box, it leaves a disturbance in the air. A mist forms around this disturbance, creating a visible trail of the particle’s path — something akin to the contrails left in the wake of an airplane.
Carl Anderson was an expert at building cloud chambers. While working for Millikan he built several, each time improving the design. He put magnets around the chamber, seeing that it caused a curvature in the particles’ paths. From the size of the curve he could deduce important information about the particles — their speed, mass and charge. He learned that an alcohol mixture worked better than water — the mist trails formed by alcohol were larger and clearer. And he placed a lead sheet over the entrance to the chamber, slowing the particles down so that they spent longer inside.
Thanks to all these innovations, Anderson was able to build a chamber far superior to any other in the world. He placed the device on the top floor of the aeronautics building at Caltech, and opened it up to the heavens. The particles came pouring in, including those unexpected visitors that seemed to move in the wrong way, apparently breaking the laws of physics. Anderson ruled out experimental error — after all he was confident in his machinery. But the question remained — what were these particles?
Scientists at the time knew of three sub-atomic particles — photons, electrons and protons. These were enough for scientists to explain the world around them. Protons and electrons make up atoms, the building blocks of all the matter in the universe. Electrons also carry electricity, lighting up cities and powering machinery. Light is carried by photons, making the world visible to our eyes.
Sub-atomic particles have properties that distinguish them, and that govern their behaviour. Two of the most important are mass and electrical charge. Mass measures how heavy a particle is. The higher the mass, the heavier the particle. The heavier the particle, the harder it is to change its direction in a magnetic field.
The other property, charge, measures how strongly a particle feels magnetic or electric forces. A particle with zero charge ignores those forces completely, passing by without feeling anything. Other particles hold either negative or positive charges — with each experiencing exactly opposite effects. When negatively-charged particles move right in a magnetic field, positively-charged particles move left.
In Anderson’s cloud chamber the mysterious particles clearly curved to the left. This had an easy explanation — protons have a positive charge, and Anderson saw several traces of protons curving left. But the curve also gives clues about mass, and these particles looked far too light to be protons. Indeed, from their mass they looked a lot more like electrons. Was it possible that Anderson had found an electron with positive, instead of negative, charge?
Anderson concluded that he had, and, after convincing his supervisor of his discovery, announced his new particle to the world. It was a bold claim, and faced immediate scepticism — but fortunately other research groups confirmed his work. The particle, the positive electron, became known as the positron, and joined the collection of sub-atomic particles. A few years later, in recognition of his work, Anderson won the Nobel Prize. At the age of 31, he was one of the youngest ever laureates.
Anderson’s discovery was welcome news to Paul Dirac, an eccentric British physicist. Years earlier he had tackled the problem of how to explain the movements of tiny and fast-moving particles, a question made relevant by studies of atoms. Scientists particularly wanted to know how atoms interacted with each other, knowledge that was vital for understanding more complicated structures like molecules. It soon turned out that electrons held the key to these interactions.
When standard quantum physics struggled to explain the way atoms behaved, some suggested that the electrons were at fault. We now know that electrons move incredibly fast inside atoms. So fast, in fact, that quantum physics is not enough — Einstein’s relativity is also needed. But Einstein’s theory only really worked for large objects, and no one, before Dirac at least, had figured out how to combine his equations with those of quantum mechanics.
Dirac started with the Schrodinger Equation, the fundamental law of quantum physics. He modified the equation to account for the time- and space-warping effects of relativity. Again and again he ran into a brick wall — the equations simply wouldn’t work out. But then, in what can only be called a moment of genius, Dirac realised that replacing the numbers in the equation with a different mathematical object could work. This object, called a matrix, solved the problem beautifully, resulting in a elegant formula that combined both relativity and quantum physics.
From the beginning Dirac’s equation presented difficulties. Although it could explain the behaviour of atoms, it also predicted many other strange things. For one thing, it said that every particle should have an oppositely charged counterpart, called an anti-particle. These particles could be created in pairs from pure energy — and if they were ever to meet they would annihilate in a tremendous flash of energy. No one had ever seen something like that happening before.
Dirac was too nice, and too well-liked, for most leading scientists to directly ridicule his theory. He was treated with pity; his theory and perceived error was called the “saddest chapter in modern physics”. Before long, rivals published another equation, one that removed many of the strange predictions made by Dirac.
The crucial error in Dirac’s work was his guess that protons and electrons formed the pair of particles predicted by his equation. Since they were the only two known charged particles they seemed like obvious candidates. But protons and electrons form atoms, and scientists calculated that putting the two so close together would cause them to annihilate in a fraction of a second. Dirac seemed to be saying that atoms, and by extension everything else, should not exist. No wonder his ideas were initially dismissed.
Anderson’s discovery, four years later, of the positron changed all that. Suddenly it was clear that protons and electrons weren’t paired — electrons and positrons were. With his theory validated, Dirac was feted by the scientific community. A year later, at the age of just 31, he was awarded the Nobel Prize. His work has since become a foundation of modern quantum mechanics, and his equation remains one of the most fundamental known to science.
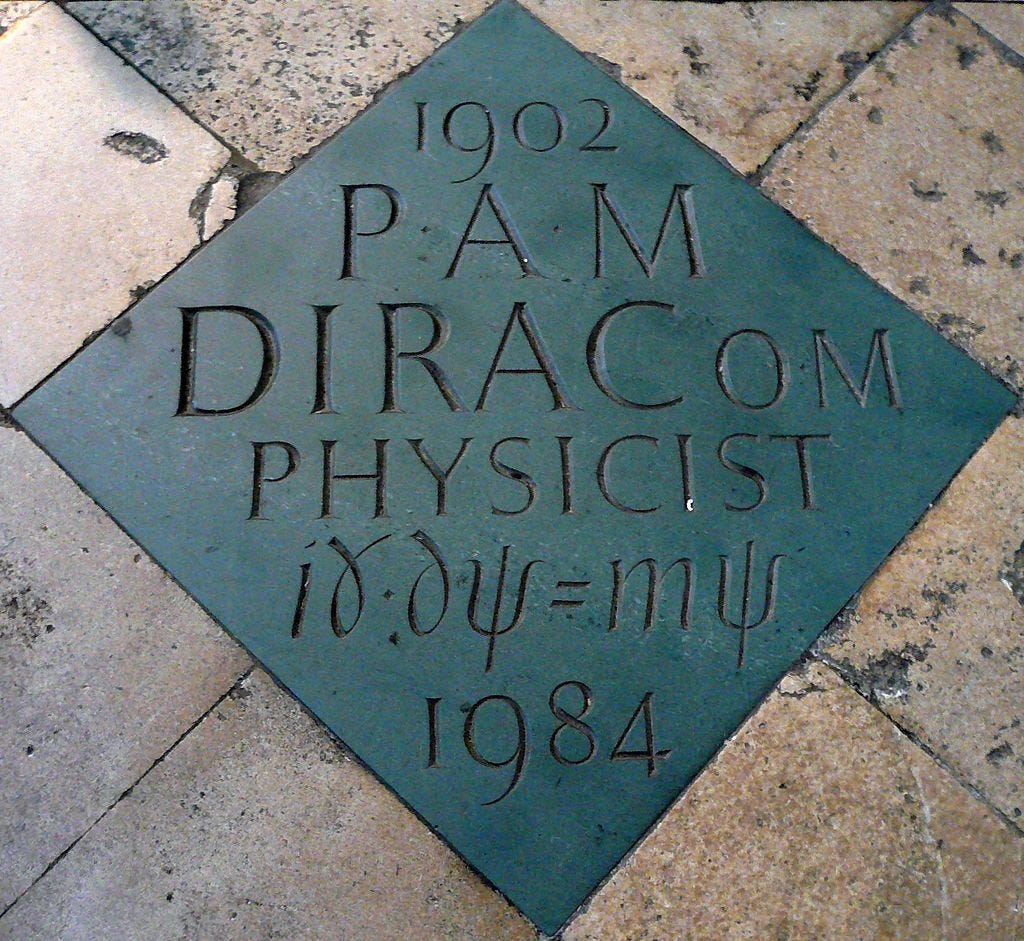
Dirac’s ideas revealed a new, hitherto unimagined, world. Everything we know, and see around us, is made out of matter. Yet, thanks to Dirac, scientists now know that another possibility exists, a world made of anti-matter. Flip all the electrons for positrons, all the protons for anti-protons, all the matter for anti-matter — and what happens? Surprisingly, it turns out that nothing much changes. An antimatter Earth would be almost identical to the real Earth. Atoms would still form, the Sun would still shine, life would still go on. The only time we need to care about matter and antimatter is when they meet, or, as it turns out, when they are created.
According to the Big Bang Theory, a single moment of creation produced everything in the Universe. First came a powerful flash of energy, from sources unknown. A few fractions of a second later, the intensity of this energy caused particles of matter and antimatter to start popping in and out of existence. In the first few milliseconds the Universe was no bigger than a speck of dust. But it quickly expanded — and as it did the energy intensity dropped. Before long the intensity would have dropped below the point at which matter and antimatter are created — bringing an end to the creation of both.
Dirac’s theory tells us that the Big Bang created exactly equal amounts of matter and antimatter — every time a matter particle was created, an antimatter partner was created with it. But Dirac also told us that matter and antimatter destroy each other, returning to pure energy. In the end, after the dust settled, the Big Bang should have resulted in equal amounts of matter and antimatter, or, if all the matter and antimatter happened to meet, in nothing at all.
Since we exist, we can rule out the second option. But the first option also doesn’t look right. The world we see is almost entirely matter. Sure, we see occasional particles of antimatter hitting the Earth from deep space, but this is a tiny amount compared to the amount of matter around. Nobody has ever found a secret well of antimatter hidden away. Miners don’t accidentally stumble upon seams of antimatter rocks, triggering tremendous explosions. Looking in the Solar System doesn’t help either. The astronauts didn’t vanish in a flash of energy when they stepped on the Moon — and neither did our space probes when they visited Mars or other planets. So where is all the antimatter?
Could it be just an accident of observation? Maybe we live in a part of the universe dominated by matter, and other regions are made of antimatter. Perhaps there are entire galaxies in far away regions of the universe, composed of swirls of anti-stars amid clouds of anti-dust and anti-gas. After all, matter and antimatter behave in very similar ways. We have no way of looking at a distant star and knowing if it is made from hydrogen or anti-hydrogen. Both look the same through a telescope.
Unfortunately, astronomers are pretty sure the whole visible universe consists of matter. If not, there should be places were matter and antimatter border, or collide. Vast amounts of energy should stream from these collisions, and they should be easily visible to our telescopes. Since we haven’t seen any sign of this, astronomers have concluded that antimatter is rare in our universe.
The evidence for the Big Bang theory is strong. But so is the evidence that matter dominates the Universe. Could there be a third option? Could, perhaps, some process favour matter over antimatter?
Historically scientists have approached such ideas with distaste. The balance between matter and anti-matter is an example of a symmetry in physics. Symmetries make for elegant maths, and physicists like elegant maths — often seeing it as evidence of a greater truth. In this case, though, a broken symmetry seems to be forced upon scientists.
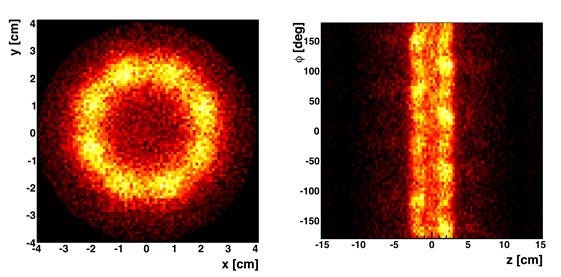
The first undeniable evidence of symmetry-breaking came in 1964. It appeared in experiments involving a group of particles known as Kaons. These are unusual particles. They undergo a process where they transform into their own antiparticles, before transforming back. But as they do this, they sometimes break apart into other particles.
In most physical processes you should end up with the same proportions of matter and antimatter you started with. But these experiments showed something different. When the Kaons broke apart, they tended to produce slightly more matter than antimatter. The effect was tiny, but it still shocked the world of physics.
Could this asymmetry explain why matter managed to survive the Big Bang, while antimatter perished? Scientists are doubtful. The effect seen in Kaons is so small that it can’t explain the amount of matter seen in the universe. Physicists have since been hunting for other examples. A handful have been found — but the imbalance is always small. So far, these asymmetries don’t seem to be enough to explain the amount of matter we see in the universe. Something else must be happening — but, whatever it is, it remains a mystery.
A century ago, when Anderson was seeing strange traces in his cloud chambers, he might have guessed he had found something interesting. But just how interesting, and the fundamental questions it would raise, he surely could never had imagined.